CYTOSKELETON NEWS – June 2023 |RhoA/ROCK in Metabolic Syndrome
はじめに
メタボリックシンドローム(MetS)またはインスリン抵抗性症候群は、心臓病、II型糖尿病、脳卒中のリスクを高める疾患です。これらの症状には、中心性肥満(すなわち、腰回りの余分な体脂肪)、高血圧、高血糖、高トリグリセリド、低HDLコレステロールなどがあります。これらの症状が3つ以上ある人は、MetSと診断される可能性があります。MetSは、米国では成人の約3分の1が罹患していると報告されています。MetSの病因はまだ完全には解明されていませんが、様々な遺伝的、環境的、生活習慣的要因が関与していると考えられています。
Rho GTPaseは、細胞骨格の調節に重要な役割を果たすシグナル伝達タンパク質ファミリーです。GTP結合によるRhoAの活性化は、アクチンの重合と安定化、ミオシンIIの機能を仲介する下流のエフェクタータンパク質の活性化につながります1。RhoAは、細胞質分裂2、ストレス線維形成3、平滑筋細胞の収縮性4などの重要な細胞内プロセスに関与しているほか、腫瘍細胞の遊走や浸潤5、心血管疾患4などの病理学的プロセスにも関与しています。RhoAとその下流のエフェクタータンパク質であるRho-associated protein kinases(ROCK1およびROCK2)もまた、MetSの発症において重要です6。ROCK活性の亢進は、肥満、インスリン抵抗性、トリグリセリド値の上昇、高血圧などのMetS病態モデルで証明されています4,7。II型糖尿病患者のPBMCでは、ROCK活性が健常対照群と比較して420-570%上昇していることが報告されています8。血清中のROCK活性は、MetS患者でも上昇しており、腰回りの周囲径、空腹時血糖値、トリグリセリド値と相関していることが示唆されました9。その結果、RhoAとROCKはMetSの治療ターゲットとして注目されています 10,11。あるランダム化比較試験では、アトルバスタチンがROCK活性を阻害することが明らかにされており、これがスタチンの抗アテローム性動脈硬化効果に寄与していることが示唆されています12。本稿では、MetSの病態に関与する生物学的プロセスにおけるRhoAとROCKシグナルの最近明らかになった役割に焦点を当てます。
RhoA/ROCKシグナルは脂肪形成におけるアクチンの細胞骨格の動態(ダイナミクス)を媒介する
脂肪細胞には主に3つのタイプがあります。
・白色脂肪細胞:余分な脂質をトリグリセリドとして蓄え、エネルギー消費量が多い時に遊離脂肪酸を放出します13。
・褐色脂肪細胞:熱を産生、つまり、エネルギーを熱に変換します14。褐色脂肪細胞は加齢とともに減少しますが、全体的な脂肪量、特に白色脂肪細胞の蓄積は加齢とともに増加します。
・ベージュ細胞:中間的な性質を持つ脂肪細胞はベージュ脂肪細胞と呼ばれ、現在のエネルギー状況に応じて白色脂肪細胞と褐色脂肪細胞の機能を切り替えることができます13,14。
褐色脂肪細胞とベージュ脂肪細胞は、エネルギーの恒常性を維持するために重要であり、それらの減少は、肥満、糖尿病、その他の代謝異常のリスク上昇と関連しています15。このため、脂肪生成プログラムを調節して発熱性脂肪細胞を有利にするシグナル伝達成分を同定することは、MetS治療の有望な戦略となりうる可能性があります。
RhoA と ROCK は、すべての代謝組織におけるアクチン細胞骨格のダイナミクスの調節と、新しい脂肪細胞を作成するプロセスである脂肪形成において重要な役割を果たしています6,16。RhoA/ROCK活性は、白色脂肪細胞とベージュ脂肪細胞の分化、および白色脂肪細胞からベージュ脂肪細胞への分化転換を阻害します6。RhoとROCKの活性は、それぞれ熱産生性の褐色脂肪細胞やベージュ脂肪細胞の分化から、筋新生や心筋細胞分化へと移行することにより、肥満をさらに促進します6。従って、RhoA/ROCK活性を標的とすることは、脂肪形成プログラムの複数のポイントに影響を与えることで、褐色脂肪細胞形成とベージュ脂肪細胞形成に移行するための手段となります。実際、マウスにおけるROCK2の阻害は、白色および褐色脂肪細胞における熱発生プログラムを促進することが報告されています17。別の研究では、ROCKシグナルの下流に位置する転写因子MRTFAを欠損させたマウスでは、食事誘発性肥満から保護され、白色脂肪組織の「ベージュ化」を示したことが実証されました18。
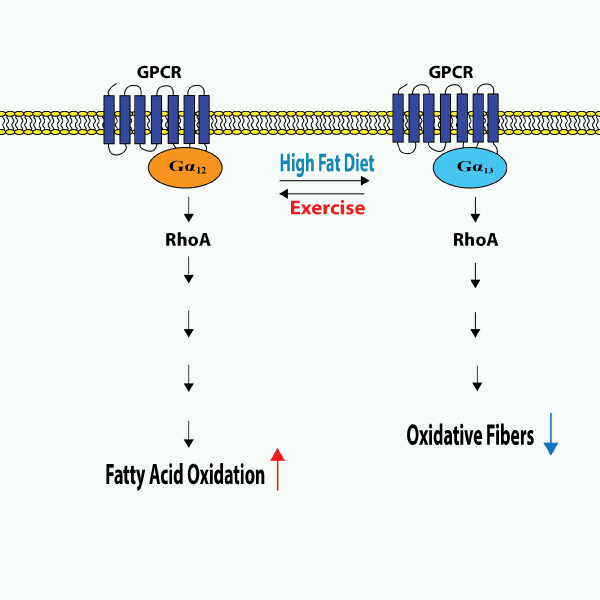
Gα12/13/RhoAは肥満、全身のエネルギー代謝、インスリン感受性、肝脂肪症に影響する
RhoAの上流では、Gα12/13サブユニットを含むヘテロ三量体Gタンパク質が、スフィンゴシン1リン酸(S1P)受容体、トロンビン受容体、プリン作動性受容体など、さまざまなGタンパク質共役型受容体(GPCR)からのシグナルを伝達します19。Gα12/13を介したGPCRシグナル伝達が広範な代謝障害に影響を及ぼしているという重要な証拠が示唆されています19,20,21。Gα12とGα13はともにRhoAの活性化につながりますが、その効果は、組織特異的に発揮され、特定の組織におけるエネルギー恒常性に関わる経路の調節において相反する役割を果たすようです19(図1)。
マウスでは、運動によって骨格筋のGα13レベルが低下する一方で、高脂肪食(HFD)摂取マウスではGα13レベルが上昇します。同様に、II型糖尿病患者の骨格筋でもGα13が上昇していることが示されています19。マウスの骨格筋特異的なGα13の除去は、全身の代謝レベルの上昇とインスリン感受性の増加につながります22。この効果は、Ser243 のリン酸化による活性化 T 細胞核因子 1 (NFATc1) の Gα13/RhoA/ROCK2 を介した抑制に依存します22。この部位でのNFATc1のリン酸化は不活性化をもたらし、肥満マウスでは上昇し、運動後には低下します23。骨格筋におけるGα13を介したRhoAの活性化はROCKを介した食事誘発性肥満につながるのに対し、Gα12を介したRhoAの活性化はHIF-1α依存性の脂肪酸酸化につながります19,22,23。HFDを与えたマウスや脂肪肝患者では、肝臓のGα12レベルが低い一方で、絶食条件下では発現が上昇します23。マウスでGα12を欠損させると、脂質の蓄積率が高くなります23。メカニズム的には、脂肪酸の酸化とミトコンドリア呼吸の調節におけるGα12のこの役割は、SIRT1/PPARα経路によって媒介されます23,24。このように、骨格筋では、Gα12/RhoAとGα13/RhoAは相反する役割を果たし、協力してエネルギー恒常性を維持しています。
NFATc1が発現していない肝臓では、この二重の制御プログラムは存在しません。骨格筋の発現レベルとは対照的に、HFD を摂取したマウスや遺伝的に肥満のマウス、および糖尿病患者では肝臓の Gα13 レベルが低くなります19。さらに、マウスの高血糖は肝臓のGα13レベルの低下を招き、耐糖能障害とインスリン抵抗性の一因となっています25。肝臓では、Gα12は肝星細胞(HSCs)におけるJNK依存性のオートファジーを促進することにより、肝臓の線維化の一因となっています19。
まとめと今後の展望 : MetSのターゲットとしてのGα12/13/RhoA/ROCK
MetSに対するこれらのシグナル伝達成分の薬理学的調節を評価する臨床試験はまだ行われていませんが、多くの前臨床動物実験が、その可能性を強調しています。その一例として、ROCKの阻害は、LKB1/AMPK経路の活性化を通じて、マウスの肥満、高コレステロール血症、耐糖能異常を抑制しました26。RhoAの下流エフェクターとそれに続く細胞プロセスは組織特異的であるため、肝臓特異的ROCK1阻害などの標的治療アプローチが前臨床マウスモデルで評価されており、その結果、エネルギー消費量の増加、インスリン感受性の向上、脂質蓄積の減少が認められました27。メタボリックシンドロームにおけるGα12/13/RhoA/ROCKシグナル伝達の多面的な役割は解明されつつあります。最近では、RhoAシグナル伝達成分をターゲットとして研究したいくつかの前臨床研究から、このシグナル伝達軸がMetSの新規ターゲットとなることが示唆されています。
■参考文献
- Toma-Fukai S, Shimizu T. Structural insights into the regulation mechanism of small GTPases by GEFs. Molecules. 2019;24(18):3308. https://doi.org/10.3390/molecules24183308.
- Paone S, Olivieri A. Role of host small GTPases in Apicomplexan parasite infection. Microorganisms. 2022;10(7):1370. https://doi.org/10.3390/microorganisms10071370.
- Corry J, Mott HR, Owen D. Activation of STAT transcription factors by the Rho-family GTPases. Biochem Soc Trans. 2020;48(5):2213–2227. https://doi.org/10.1042/bst20200468.
- Cherfils J, Zeghouf M. Regulation of small GTPases by GEFs, GAPs, and GDIs. Physiol Rev. 2013;93(1):269–309. https://doi.org/10.1152/physrev.00003.2012.
- Rottner K, Stradal TEB, Wehland J. Bacteria–host-cell interactions at the plasma membrane: Stories on actin cytoskeleton subversion. Dev Cell. 2005;9(1):3–17. https://doi.org/10.1016/j.devcel.2005.06.002.
- Higgs HN, Pollard TD. Activation by Cdc42 and PIP2 of Wiskott–Aldrich syndrome protein (WASP) stimulates actin nucleation by Arp2/3 complex. J Cell Biol. 2001;150(6):1311–1320. https://doi.org/10.1083/jcb.150.6.1311.
- Tomasevic N, Jia Z, Russell A, Fujii T, Hartman JJ, Clancy S, Wang M, Beraud C, Wood KW, Sakowicz R. Differential regulation of WASP and N-WASP by Cdc42, Rac1, Nck, and PI(4,5)P2. Biochemistry. 2007;46(11):3494–3502. https://doi.org/10.1021/bi062152y.
- Ding B, Yang S, Schaks M, Liu Y, Brown AJ, Rottner K, Chowdhury S, Chen B. Structures reveal a key mechanism of WAVE regulatory complex activation by Rac1 GTPase. Nat Commun. 2022;13(1):5444. https://doi.org/10.1038/s41467-022-33174-3.
- Boehm M, Krause-Gruszcyznska M, Rohde M, Tegtmeyer N, Takahashi S, Oyarzabal OA, Backert S. Major host factors involved in epithelial cell invasion of Campylobacter jejuni: Role of fibronectin, integrin β1, FAK, Tiam-1, and DOCK180 in activating Rho GTPase Rac1. Front Cell Infect Microbiol. 2011;1:17. https://doi.org/10.3389/fcimb.2011.00017.
- Friebel A, Ilchmann H, Aepfelbacher M, Ehrbar K, Machleidt W, Hardt WD. SopE and SopE2 from Salmonella typhimurium activate different sets of RhoGTPases of the host cell. J Biol Chem. 2001;276(36):34035–34040. https://doi.org/10.1074/jbc.m100609200.
- Humphreys D, Davidson A, Hume PJ, Koronakis V. Salmonella virulence effector SopE and host GEF ARNO cooperate to recruit and activate WAVE to trigger bacterial invasion. Cell Host Microbe. 2012;11(2):129–139. https://doi.org/10.1016/j.chom.2012.01.006.
- Singh V, Davidson AC, Hume PJ, Humphreys D, Koronakis V. Arf GTPase interplay with Rho GTPases in regulation of the actin cytoskeleton. Small GTPases. 2019;10(6):411–418. https://doi.org/10.1080/21541248.2017.1329691.
- Nichols CD, Casanova JE. Salmonella-directed recruitment of new membrane to invasion foci via the host exocyst complex. Curr Biol. 2010;20(14):1316–1320. https://doi.org/10.1016/j.cub.2010.05.065.
- Galán JE, Zhou D. Striking a balance: Modulation of the actin cytoskeleton by Salmonella. Proc Natl Sci U S A. 2000;97(16):8754–8761. https://doi.org/10.1073/pnas.97.16.8754.
- Stebbins CE, Galán JE. Modulation of host signaling by a bacterial mimic: Structure of the Salmonella effector SptP bound to Rac1. Mol Cell. 2000;6(6):1449–1460. https://doi.org/10.1016/s1097-2765(00)00141-6.
- Knuff K, Finlay BB. What the SIF is happening—the role of intracellular Salmonella-induced filaments. Front Cell Infect Microbiol. 2017;7:335. https://doi.org/10.3389/fcimb.2017.00335.
- McEwan DG, Richter B, Claudi B, Wigge C, Wild P, Farhan H, McGourty K, Coxon FP, Franz-Wachtel M, Perdu B, Akutsu M, Habermann A, Kirchof A, Helfrich MH, Odgren PR, Van Hul W, Frangakis AS, Rajalingam K, Macek B, Holden DW, Bumann D, Dikic I. PLEKHM1 regulates Salmonella-containing vacuole biogenesis and infection. Cell Host Microbe. 2015;17(1):58–71. https://doi.org/10.1016/j.chom.2014.11.011.
- McGourty K, Thurston TL, Matthews SA, Pinaud L, Mota LJ, Holden DW. Salmonella inhibits retrograde trafficking of mannose-6-phosphate receptors and lysosome function. Science. 2012;338(6109):963–967. https://doi.org/10.1126/science.1227037.
- Bugalhão JN, Mota LJ. The multiple functions of the numerous Chlamydia trachomatis secreted proteins: The tip of the iceberg. Microb Cell. 2019;6(9):414–449. https://doi.org/10.15698/mic2019.09.691.
- Haines A, Wesolowski J, Ryan NM, Monteiro-Brás T, Paumet F. Cross talk between ARF1 and RhoA coordinates the formation of cytoskeletal scaffolds during Chlamydia infection. mBio. 2021;12(6):e0239721. https://doi.org/10.1128/mbio.02397-21.
- Al-Zeer MA, Al-Younes HM, Kerr M, Abu-Lubad M, Gonzalez E, Brinkmann V, Meyer TF. Chlamydia trachomatis remodels stable microtubules to coordinate Golgi stack recruitment to the chlamydial inclusion surface. Mol Microbiol. 2014;94(6):1285–1297. https://doi.org/10.1111/mmi.12829.
- Faris R, Merling M, Andersen SE, Dooley CA, Hackstadt T, Weber MM. Chlamydia trachomatis CT229 subverts Rab GTPase-dependent CCV trafficking pathways to promote chlamydial infection. Cell Rep. 2019;26(12):3380–3390. https://doi.org/10.1016/j.celrep.2019.02.079.
- Ao X, Zou L, Wu Y. Regulation of autophagy by the Rab GTPase network. Cell Death Differ. 2014;21(3):348–358. https://doi.org/10.1038/cdd.2013.187.
- Thomas DR, Newton P, Lau N, Newton HJ. Interfering with autophagy: The opposing strategies deployed by Legionella pneumophila and Coxiella burnetii effector proteins. Front Cell Infect Microbiol. 2020;10:599762. https://doi.org/10.3389/fcimb.2020.599762.
- Dong W, He B, Qian H, Lu Q, Wang D, Li J, Wei Z, Wang Z, Xu Z, Wu G, Qian G, Wang G. RAB26-dependent autophagy protects adherens junctional integrity in acute lung injury. Autophagy. 2018;14(10):1677–1692. https://doi.org/10.1080/15548627.2018.1476811.
- Liu H, Zhou Y, Qiu H, Zhuang R, Han Y, Liu X, Qiu X, Wang Z, Xu L, Tan R, Hong W, Wang T, Rab26 suppresses migration and invasion of breast cancer cells through mediating autophagic degradation of phosphorylated Src. Cell Death Dis. 2021;12(4):284. https://doi.org/10.1038/s41419-021-03561-7.
- Ma Z, Liu K, Zhang R-F, Xie Z-X, Liu W, Deng Y, Li X, Xu B. Manganese-induced α-synuclein overexpression promotes the accumulation of dysfunctional synaptic vesicles and hippocampal synaptotoxicity by suppressing Rab26-dependent autophagy in presynaptic neurons. Sci Total Environ. 2023;858:159753. https://doi.org/10.1016/j.scitotenv.2022.159753.
- Tominello TR, Oliviera ERA, Hussain SS, Elfert A, Wells J, Golden B, Ismail N. Emerging roles of autophagy and inflammasome in ehrlichiosis. Front Immunol. 2019;10:1011. https://doi.org/10.3389/fimmu.2019.01011.
- Lebreton A, Stavru F, Cossart P. Organelle targeting during bacterial infection: insights from Listeria. Trends Cell Biol. 2015;25(6):330–338. https://doi.org/10.1016/j.tcb.2015.01.003.
- Huang J, Brumell JH. Bacteria–autophagy interplay: A battle for survival. Nat Rev Microbiol. 2014;12(2):101–114. https://doi.org/10.1038/nrmicro3160.
- Charro N, Mota LJ. Approaches targeting the type III secretion system to treat or prevent bacterial infections. Expert Opin Drug Discov. 2015;10(4):373–387. https://doi.org/10.1517/17460441.2015.1019860.
- Hotinger JA, Morris ST, May AE. The case against antibiotics and for anti-virulence therapeutics. Microorganisms. 2021;9(10):2049. https://doi.org/10.3390/microorganisms9102049.
- Hu X, Yu J, Zhou X, Li Z, Xia Y, Luo Z, Wu Y. A small GTPase‑like protein fragment of Mycoplasma promotes tumor cell migration and proliferation in vitro via interaction with Rac1 and Stat3. Mol Med Rep. 2014;9(1):173–179. https://doi.org/10.3892/mmr.2013.1766.
- Nicolson GL. Pathogenic Mycoplasma infections in chronic illnesses: General considerations in selecting conventional and integrative treatments. Int J Clin Med. 2019;10(10):477–522.
関連商品
活性型低分子量Gタンパク質 プルダウンアッセイ Biochem Kit™
Cytoskeleton(サイトスケルトン)社 CYTOSKELETON NEWS
※コスモ・バイオのホームページ上にあるCYTOSKELETON NEWSの特集ページです。
掲載元:Cytoskeleton News 2023年6月号